Room temperature manipulation of long lifetime spins in metallic-like carbon nanospheres
- PMID: 27426851
- PMCID: PMC4960311
- DOI: 10.1038/ncomms12232
Room temperature manipulation of long lifetime spins in metallic-like carbon nanospheres
Abstract
The time-window for processing electron spin information (spintronics) in solid-state quantum electronic devices is determined by the spin-lattice and spin-spin relaxation times of electrons. Minimizing the effects of spin-orbit coupling and the local magnetic contributions of neighbouring atoms on spin-lattice and spin-spin relaxation times at room temperature remain substantial challenges to practical spintronics. Here we report conduction electron spin-lattice and spin-spin relaxation times of 175 ns at 300 K in 37±7 nm carbon spheres, which is remarkably long for any conducting solid-state material of comparable size. Following the observation of spin polarization by electron spin resonance, we control the quantum state of the electron spin by applying short bursts of an oscillating magnetic field and observe coherent oscillations of the spin state. These results demonstrate the feasibility of operating electron spins in conducting carbon nanospheres as quantum bits at room temperature.
Figures
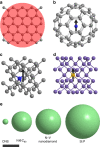
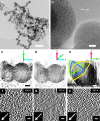
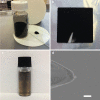
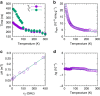
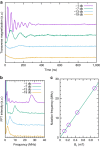
Similar articles
-
Driven coherent oscillations of a single electron spin in a quantum dot.Nature. 2006 Aug 17;442(7104):766-71. doi: 10.1038/nature05065. Nature. 2006. PMID: 16915280
-
Readout and control of a single nuclear spin with a metastable electron spin ancilla.Nat Nanotechnol. 2013 Jul;8(7):487-92. doi: 10.1038/nnano.2013.104. Epub 2013 Jun 23. Nat Nanotechnol. 2013. PMID: 23793305
-
Sensing Coherent Dynamics of Electronic Spin Clusters in Solids.Phys Rev Lett. 2018 Jun 15;120(24):243604. doi: 10.1103/PhysRevLett.120.243604. Phys Rev Lett. 2018. PMID: 29956999
-
Coherent dynamics of coupled electron and nuclear spin qubits in diamond.Science. 2006 Oct 13;314(5797):281-5. doi: 10.1126/science.1131871. Epub 2006 Sep 14. Science. 2006. PMID: 16973839
-
Coherent spin manipulation without magnetic fields in strained semiconductors.Nature. 2004 Jan 1;427(6969):50-3. doi: 10.1038/nature02202. Nature. 2004. PMID: 14702080
Cited by
-
A dual spin-controlled chiral two-/three-dimensional perovskite artificial leaf for efficient overall photoelectrochemical water splitting.Nat Commun. 2024 Jun 1;15(1):4672. doi: 10.1038/s41467-024-49216-x. Nat Commun. 2024. PMID: 38824151 Free PMC article.
-
Room-temperature photonic logical qubits via second-order nonlinearities.Nat Commun. 2021 Jan 8;12(1):191. doi: 10.1038/s41467-020-20417-4. Nat Commun. 2021. PMID: 33420052 Free PMC article.
-
Long-lived electronic spin qubits in single-walled carbon nanotubes.Nat Commun. 2023 Feb 15;14(1):848. doi: 10.1038/s41467-023-36031-z. Nat Commun. 2023. PMID: 36792597 Free PMC article.
References
-
- DiVincenzo D. P. Quantum computation. Science 270, 255 (1995).
-
- Poole C. P. J. & Farach H. A. Relaxation in Magnetic Resonance Academic Press (1971).
-
- Bloch F. Nuclear induction. Phys. Rev. 70, 460 (1946).
-
- Jánossy A. Resonant and nonresonant conduction-electron-spin transmission in normal metals. Phys. Rev. B 21, 3793–3810 (1980).
-
- Ardavan A. et al.. Will spin-relaxation times in molecular magnets permit quantum information processing? Phys. Rev. Lett. 98, 057201 (2007). - PubMed
Publication types
LinkOut - more resources
Full Text Sources
Other Literature Sources
Miscellaneous