Pteropods make thinner shells in the upwelling region of the California Current Ecosystem
- PMID: 33462349
- PMCID: PMC7814018
- DOI: 10.1038/s41598-021-81131-9
Pteropods make thinner shells in the upwelling region of the California Current Ecosystem
Abstract
Shelled pteropods are widely regarded as bioindicators for ocean acidification, because their fragile aragonite shells are susceptible to increasing ocean acidity. While short-term incubations have demonstrated that pteropod calcification is negatively impacted by ocean acidification, we know little about net calcification in response to varying ocean conditions in natural populations. Here, we examine in situ calcification of Limacina helicina pteropods collected from the California Current Ecosystem, a coastal upwelling system with strong spatial gradients in ocean carbonate chemistry, dissolved oxygen and temperature. Depth-averaged pH ranged from 8.03 in warmer offshore waters to 7.77 in cold CO2-rich waters nearshore. Based on high-resolution micro-CT technology, we showed that shell thickness declined by ~ 37% along the upwelling gradient from offshore to nearshore water. Dissolution marks covered only ~ 2% of the shell surface area and were not associated with the observed variation in shell thickness. We thus infer that pteropods make thinner shells where upwelling brings more acidified and colder waters to the surface. Probably the thinner shells do not result from enhanced dissolution, but are due to a decline in calcification. Reduced calcification of pteropods is likely to have major ecological and biogeochemical implications for the cycling of calcium carbonate in the oceans.
Conflict of interest statement
The authors declare no competing interests.
Figures
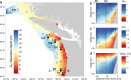
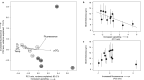
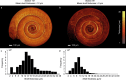
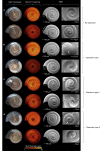
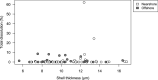
Similar articles
-
Limacina helicina shell dissolution as an indicator of declining habitat suitability owing to ocean acidification in the California Current Ecosystem.Proc Biol Sci. 2014 Apr 30;281(1785):20140123. doi: 10.1098/rspb.2014.0123. Print 2014 Jun 22. Proc Biol Sci. 2014. PMID: 24789895 Free PMC article.
-
Dissolution dominating calcification process in polar pteropods close to the point of aragonite undersaturation.PLoS One. 2014 Oct 6;9(10):e109183. doi: 10.1371/journal.pone.0109183. eCollection 2014. PLoS One. 2014. PMID: 25285916 Free PMC article.
-
Shell condition and survival of Puget Sound pteropods are impaired by ocean acidification conditions.PLoS One. 2014 Aug 27;9(8):e105884. doi: 10.1371/journal.pone.0105884. eCollection 2014. PLoS One. 2014. PMID: 25162395 Free PMC article.
-
Evolution and biomineralization of pteropod shells.J Struct Biol. 2021 Dec;213(4):107779. doi: 10.1016/j.jsb.2021.107779. Epub 2021 Aug 30. J Struct Biol. 2021. PMID: 34474158 Review.
-
Ocean acidification and its potential effects on marine ecosystems.Ann N Y Acad Sci. 2008;1134:320-42. doi: 10.1196/annals.1439.013. Ann N Y Acad Sci. 2008. PMID: 18566099 Review.
Cited by
-
Pelagic calcium carbonate production and shallow dissolution in the North Pacific Ocean.Nat Commun. 2023 Feb 20;14(1):805. doi: 10.1038/s41467-023-36177-w. Nat Commun. 2023. PMID: 36808154 Free PMC article.
-
The impacts of past, present and future ocean chemistry on predatory planktonic snails.R Soc Open Sci. 2021 Aug 4;8(8):202265. doi: 10.1098/rsos.202265. eCollection 2021 Aug. R Soc Open Sci. 2021. PMID: 34386247 Free PMC article.
-
Contrasting patterns in pH variability in the Arabian Sea and Bay of Bengal.Environ Sci Pollut Res Int. 2024 Feb;31(10):15271-15288. doi: 10.1007/s11356-024-31950-w. Epub 2024 Jan 30. Environ Sci Pollut Res Int. 2024. PMID: 38289549
-
Aragonite dissolution protects calcite at the seafloor.Nat Commun. 2022 Mar 1;13(1):1104. doi: 10.1038/s41467-022-28711-z. Nat Commun. 2022. PMID: 35232971 Free PMC article.
-
Sea butterflies in a pickle: reliable biomarkers and seasonal sensitivity of Limacina retroversa to ocean acidification in the Gulf of Maine.Conserv Physiol. 2024 Jun 21;12(1):coae040. doi: 10.1093/conphys/coae040. eCollection 2024. Conserv Physiol. 2024. PMID: 38915852 Free PMC article.
References
-
- Friedlingstein P, et al. Global carbon budget 2019. Earth Syst. Sci. Data. 2019;11:1783–1838. doi: 10.5194/essd-11-1783-2019. - DOI
Publication types
LinkOut - more resources
Full Text Sources
Other Literature Sources