Evidence for a liquid silicate layer atop the Martian core
- PMID: 37880439
- PMCID: PMC10600012
- DOI: 10.1038/s41586-023-06586-4
Evidence for a liquid silicate layer atop the Martian core
Abstract
Seismic recordings made during the InSight mission1 suggested that Mars's liquid core would need to be approximately 27% lighter than pure liquid iron2,3, implying a considerable complement of light elements. Core compositions based on seismic and bulk geophysical constraints, however, require larger quantities of the volatile elements hydrogen, carbon and sulfur than those that were cosmochemically available in the likely building blocks of Mars4. Here we show that multiply diffracted P waves along a stratified core-mantle boundary region of Mars in combination with first-principles computations of the thermoelastic properties of liquid iron-rich alloys3 require the presence of a fully molten silicate layer overlying a smaller, denser liquid core. Inverting differential body wave travel time data with particular sensitivity to the core-mantle boundary region suggests a decreased core radius of 1,675 ± 30 km associated with an increased density of 6.65 ± 0.1 g cm-3, relative to previous models2,4-8, while the thickness and density of the molten silicate layer are 150 ± 15 km and 4.05 ± 0.05 g cm-3, respectively. The core properties inferred here reconcile bulk geophysical and cosmochemical requirements, consistent with a core containing 85-91 wt% iron-nickel and 9-15 wt% light elements, chiefly sulfur, carbon, oxygen and hydrogen. The chemical characteristics of a molten silicate layer above the core may be revealed by products of Martian magmatism.
© 2023. The Author(s).
Conflict of interest statement
The authors declare no competing interests.
Figures
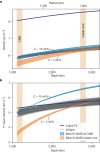
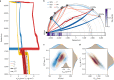
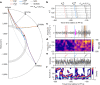
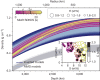
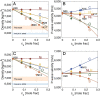
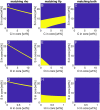
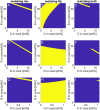
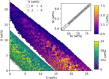
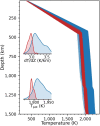
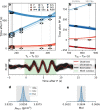
Similar articles
-
Geophysical evidence for an enriched molten silicate layer above Mars's core.Nature. 2023 Oct;622(7984):712-717. doi: 10.1038/s41586-023-06601-8. Epub 2023 Oct 25. Nature. 2023. PMID: 37880437 Free PMC article.
-
First observations of core-transiting seismic phases on Mars.Proc Natl Acad Sci U S A. 2023 May 2;120(18):e2217090120. doi: 10.1073/pnas.2217090120. Epub 2023 Apr 24. Proc Natl Acad Sci U S A. 2023. PMID: 37094138 Free PMC article.
-
Core Formation and Geophysical Properties of Mars.Earth Planet Sci Lett. 2020 Jan 15;530:115923. doi: 10.1016/j.epsl.2019.115923. Epub 2019 Nov 11. Earth Planet Sci Lett. 2020. PMID: 32647387 Free PMC article.
-
Seismic detection of the martian core.Science. 2021 Jul 23;373(6553):443-448. doi: 10.1126/science.abi7730. Science. 2021. PMID: 34437118
-
Deep penetration of molten iron into the mantle caused by a morphological instability.Nature. 2012 Dec 13;492(7428):243-6. doi: 10.1038/nature11663. Nature. 2012. PMID: 23235879
Cited by
-
A thermally conductive Martian core and implications for its dynamo cessation.Sci Adv. 2024 Mar 22;10(12):eadk1087. doi: 10.1126/sciadv.adk1087. Epub 2024 Mar 20. Sci Adv. 2024. PMID: 38507495 Free PMC article.
-
Weak magnetism of Martian impact basins may reflect cooling in a reversing dynamo.Nat Commun. 2024 Aug 9;15(1):6831. doi: 10.1038/s41467-024-51092-4. Nat Commun. 2024. PMID: 39122701 Free PMC article.
-
Mars has a surprise layer of molten rock inside.Nature. 2023 Nov;623(7985):20. doi: 10.1038/d41586-023-03271-4. Nature. 2023. PMID: 37880531 No abstract available.
-
Marsquakes shake up views of the Red Planet's deep interior.Proc Natl Acad Sci U S A. 2024 Feb 6;121(6):e2400778121. doi: 10.1073/pnas.2400778121. Epub 2024 Feb 2. Proc Natl Acad Sci U S A. 2024. PMID: 38306479 Free PMC article. No abstract available.
References
-
- Banerdt WB, et al. Initial results from the InSight mission on Mars. Nat. Geosci. 2020;13:183–189. doi: 10.1038/s41561-020-0544-y. - DOI
-
- Huang D, et al. Thermoelastic properties of liquid fe-rich alloys under martian core conditions. Geophys. Res. Lett. 2023;50:e2022GL102271. doi: 10.1029/2022GL102271. - DOI
-
- Khan A, Sossi P, Liebske C, Rivoldini A, Giardini D. Geophysical and cosmochemical evidence for a volatile-rich Mars. Earth Planet. Sci. Lett. 2022;578:117330. doi: 10.1016/j.epsl.2021.117330. - DOI
LinkOut - more resources
Full Text Sources
Miscellaneous