High-Performance Multiwavelength GaNAs Single Nanowire Lasers
- PMID: 38166147
- PMCID: PMC10795468
- DOI: 10.1021/acsnano.3c07980
High-Performance Multiwavelength GaNAs Single Nanowire Lasers
Abstract
In this study, we report a significant enhancement in the performance of GaNAs-based single nanowire lasers through optimization of growth conditions, leading to a lower lasing threshold and higher operation temperatures. Our analysis reveals that these improvements in the laser performance can be attributed to a decrease in the density of localized states within the material. Furthermore, we demonstrate that owing to their excellent nonlinear optical properties, these nanowires support self-frequency conversion of the stimulated emission through second harmonic generation (SHG) and sum-frequency generation (SFG), providing coherent light emission in the cyan-green range. Mode-specific differences in the self-conversion efficiency are revealed and explained by differences in the light extraction efficiency of the converted light caused by the electric field distribution of the fundamental modes. Our work, therefore, facilitates the design and development of multiwavelength coherent light generation and higher-temperature operation of GaNAs nanowire lasers, which will be useful in the fields of optical communications, sensing, and nanophotonics.
Keywords: coherent light; lasing; multiwavelength coherent light; nanophotonics; nanowires; nonlinear optics; second harmonic generation (SHG).
Conflict of interest statement
The authors declare no competing financial interest.
Figures
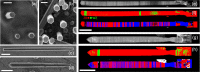
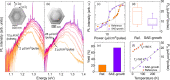
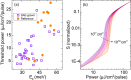
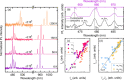
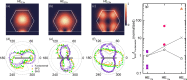
Similar articles
-
Direct visualization of phase-matched efficient second harmonic and broadband sum frequency generation in hybrid plasmonic nanostructures.Light Sci Appl. 2020 Oct 22;9:180. doi: 10.1038/s41377-020-00414-4. eCollection 2020. Light Sci Appl. 2020. PMID: 33110598 Free PMC article.
-
Dilute Nitride Nanowire Lasers Based on a GaAs/GaNAs Core/Shell Structure.Nano Lett. 2017 Mar 8;17(3):1775-1781. doi: 10.1021/acs.nanolett.6b05097. Epub 2017 Feb 8. Nano Lett. 2017. PMID: 28170267
-
Near-Infrared Lasing at 1 μm from a Dilute-Nitride-Based Multishell Nanowire.Nano Lett. 2019 Feb 13;19(2):885-890. doi: 10.1021/acs.nanolett.8b04103. Epub 2019 Jan 9. Nano Lett. 2019. PMID: 30608174
-
Single-Mode Semiconductor Nanowire Lasers With Coupled Cavities.Front Chem. 2021 Jan 15;8:631870. doi: 10.3389/fchem.2020.631870. eCollection 2020. Front Chem. 2021. PMID: 33520944 Free PMC article. Review.
-
ZnO nanowire lasers.Nanoscale. 2011 Jul;3(7):2783-800. doi: 10.1039/c1nr00013f. Epub 2011 May 6. Nanoscale. 2011. PMID: 21552596 Review.
References
-
- Schmiedeke P.; Thurn A.; Matich S.; Döblinger M.; Finley J. J.; Koblmüller G. Low-Threshold Strain-Compensated InGaAs/(In,Al)GaAs Multi-Quantum Well Nanowire Lasers Emitting near 1.3 μm at Room Temperature. Appl. Phys. Lett. 2021, 118 (22), 22110310.1063/5.0048807. - DOI