N-glycosylation as a eukaryotic protective mechanism against protein aggregation
- PMID: 38295165
- PMCID: PMC10830103
- DOI: 10.1126/sciadv.adk8173
N-glycosylation as a eukaryotic protective mechanism against protein aggregation
Abstract
The tendency for proteins to form aggregates is an inherent part of every proteome and arises from the self-assembly of short protein segments called aggregation-prone regions (APRs). While posttranslational modifications (PTMs) have been implicated in modulating protein aggregation, their direct role in APRs remains poorly understood. In this study, we used a combination of proteome-wide computational analyses and biophysical techniques to investigate the potential involvement of PTMs in aggregation regulation. Our findings reveal that while most PTM types are disfavored near APRs, N-glycosylation is enriched and evolutionarily selected, especially in proteins prone to misfolding. Experimentally, we show that N-glycosylation inhibits the aggregation of peptides in vitro through steric hindrance. Moreover, mining existing proteomics data, we find that the loss of N-glycans at the flanks of APRs leads to specific protein aggregation in Neuro2a cells. Our findings indicate that, among its many molecular functions, N-glycosylation directly prevents protein aggregation in higher eukaryotes.
Figures
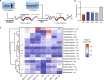
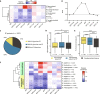
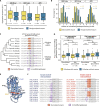
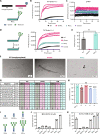
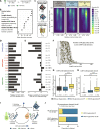
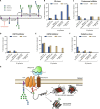
Similar articles
-
Aggregation prone regions in human proteome: Insights from large-scale data analyses.Proteins. 2017 Jun;85(6):1099-1118. doi: 10.1002/prot.25276. Epub 2017 Mar 24. Proteins. 2017. PMID: 28257595
-
Proteome-Wide Analysis of N-Glycosylation Stoichiometry Using SWATH Technology.J Proteome Res. 2017 Oct 6;16(10):3830-3840. doi: 10.1021/acs.jproteome.7b00480. Epub 2017 Sep 15. J Proteome Res. 2017. PMID: 28845672
-
Aggregating sequences that occur in many proteins constitute weak spots of bacterial proteostasis.Nat Commun. 2018 Feb 28;9(1):866. doi: 10.1038/s41467-018-03131-0. Nat Commun. 2018. PMID: 29491361 Free PMC article.
-
The Methods Employed in Mass Spectrometric Analysis of Posttranslational Modifications (PTMs) and Protein-Protein Interactions (PPIs).Adv Exp Med Biol. 2019;1140:169-198. doi: 10.1007/978-3-030-15950-4_10. Adv Exp Med Biol. 2019. PMID: 31347048 Free PMC article. Review.
-
Identification, Quantification, and Site Localization of Protein Posttranslational Modifications via Mass Spectrometry-Based Proteomics.Adv Exp Med Biol. 2016;919:345-382. doi: 10.1007/978-3-319-41448-5_17. Adv Exp Med Biol. 2016. PMID: 27975226 Review.
Cited by
-
N-glycosylation in Archaea - Expanding the process, components and roles of a universal post-translational modification.BBA Adv. 2024 Aug 29;6:100120. doi: 10.1016/j.bbadva.2024.100120. eCollection 2024. BBA Adv. 2024. PMID: 39296579 Free PMC article.
-
Glycosylation of serine/threonine-rich intrinsically disordered regions of membrane-associated proteins in streptococci.bioRxiv [Preprint]. 2025 Mar 17:2024.05.05.592596. doi: 10.1101/2024.05.05.592596. bioRxiv. 2025. PMID: 38746434 Free PMC article. Preprint.
-
Investigating Medin Cleavage Accessibility in MfgE8: Conformational Insights Derived from Molecular Dynamics Simulations and AlphaFold2 Models.bioRxiv [Preprint]. 2024 Jul 29:2024.07.27.605412. doi: 10.1101/2024.07.27.605412. bioRxiv. 2024. PMID: 39131300 Free PMC article. Preprint.
References
-
- Fernandez-Escamilla A. M., Rousseau F., Schymkowitz J., Serrano L., Prediction of sequence-dependent and mutational effects on the aggregation of peptides and proteins. Nat. Biotechnol. 22, 1302–1306 (2004). - PubMed
-
- Rousseau F., Serrano L., Schymkowitz J. W. H., How evolutionary pressure against protein aggregation shaped chaperone specificity. J. Mol. Biol. 355, 1037–1047 (2006). - PubMed
-
- Prabakaran R., Goel D., Kumar S., Gromiha M. M., Aggregation prone regions in human proteome: Insights from large-scale data analyses. Proteins 85, 1099–1118 (2017). - PubMed
-
- Tyedmers J., Mogk A., Bukau B., Cellular strategies for controlling protein aggregation. Nat. Rev. Mol. Cell Biol. 11, 777–788 (2010). - PubMed
MeSH terms
Substances
LinkOut - more resources
Full Text Sources
Miscellaneous